Dinoflagellates emit bioluminescent light to survive from predators through a complex biochemical cascade, currently being investigated by Dr. Michael Latz at UC San Diego.
When was the last time you looked up at the night sky and were amazed by the spectacular scene of distant stars thousands of times larger than our sun? How about when you last looked down into the ocean to see an even more glamorous—albeit microscopic—shade of neon blue created by microorganisms hundreds of times smaller than a grain of rice?
Bioluminescence, or light produced by living organisms, has been observed for eons and has fascinated scientists for many centuries. Organisms like jellyfish, shrimp, and zooplankton acquired this ability and started to use it to defend themselves against predators, attract mates, and hide themselves in the deep ocean. Notably, one of the most heavenly sights to see here in La Jolla, California has to be our neon blue tide. However, we must not forget to thank the group of organisms that emit this gorgeous light—
dinoflagellates.
Shining to Live
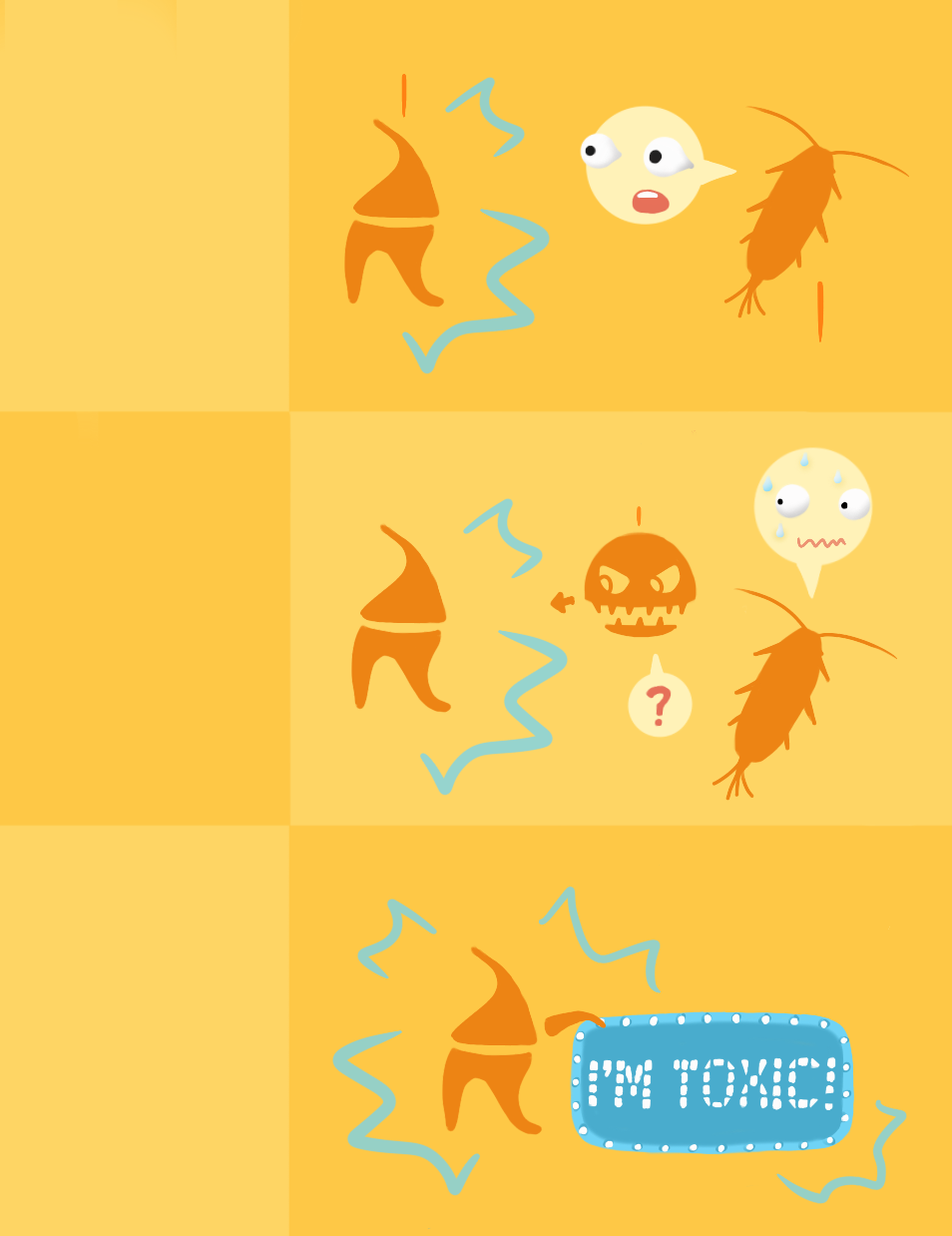
Three theories on how dinoflagellates use bioluminescence to escape from copepods.
Dinoflagellates are single-cell aquatic phytoplankton that usually emit light at night to avoid predators like copepods.¹ Copepods are the most abundant zoo-plankton in the ocean, and therefore exert top-down control on the population of dinoflagellates. Top-down control refers to top consumers, or the predators in a food web, controlling the population distribution of lower trophic levels, or the prey. Just like cows on land, copepods have grazing behavior; however, instead of grass, they graze on phytoplankton like dinoflagellates. Thus far, there are three major hypotheses describing how dinoflagellates utilize bioluminescence to evade copepod predation.
The first hypothesis is that dinoflagellates produce a flash of light to startle copepods. This light interrupts copepod grazing behavior, allowing dinoflagellates time to escape from their predator.²
In addition, high light intensities make copepods swim faster and straighter, potentially forcing them to swim away from an area with a large density of dinoflagellates. It has been observed that copepod swimming behavior is indeed affected by natural and artificial dinoflagellate flashes, making this theory very persuasive.¹ Interestingly, only marine and estuarine copepods exposed to dinoflagellates in their environment respond to light flashes with increased burst swimming—freshwater copepods do not respond to dinoflagellate flashes. This observation indicates that the marine copepods’ light-evasive burst swimming behavior evolved separately from their relatives in other environments. The divergence could be due to the exclusive presence of bioluminescent dinoflagellates in marine environments and the fact that copepods’ visual predators attack them more often in the light than in the dark.
Another popular theory on how bioluminescence enhances dinoflagellate evasion from copepod predation is that their blue flashes of light may attract copepods’ predators. It has been observed that lipid compounds released by copepods cause certain species of dinoflagellates to emit high-intensity light even at low colony densities.¹ In this way, visual predators like fish or squid might be attracted by the bright light to feed on the copepods. As a result, not only do dinoflagellates remain uneaten and their predators are removed, but the copepods’ ability to habituate to the light flash is also minimized, since the copepods who adapt and do not swim away have a higher chance of being eaten by their predators.
The third theory hypothesizes that bioluminescence serves as an aposematic—or warning—signal indicating that dinoflagellates are toxic to predators. There are about twelve species of bioluminescent dinoflagellates that are known to be toxic to copepods when ingested. However, though their colors might be a very effective aposematic signal, it is not clear if warning predators is the main purpose of bioluminescence, as copepods can often distinguish between toxic and non-toxic dinoflagellates using other visual means or chemical sensing.³
Excitement for Light
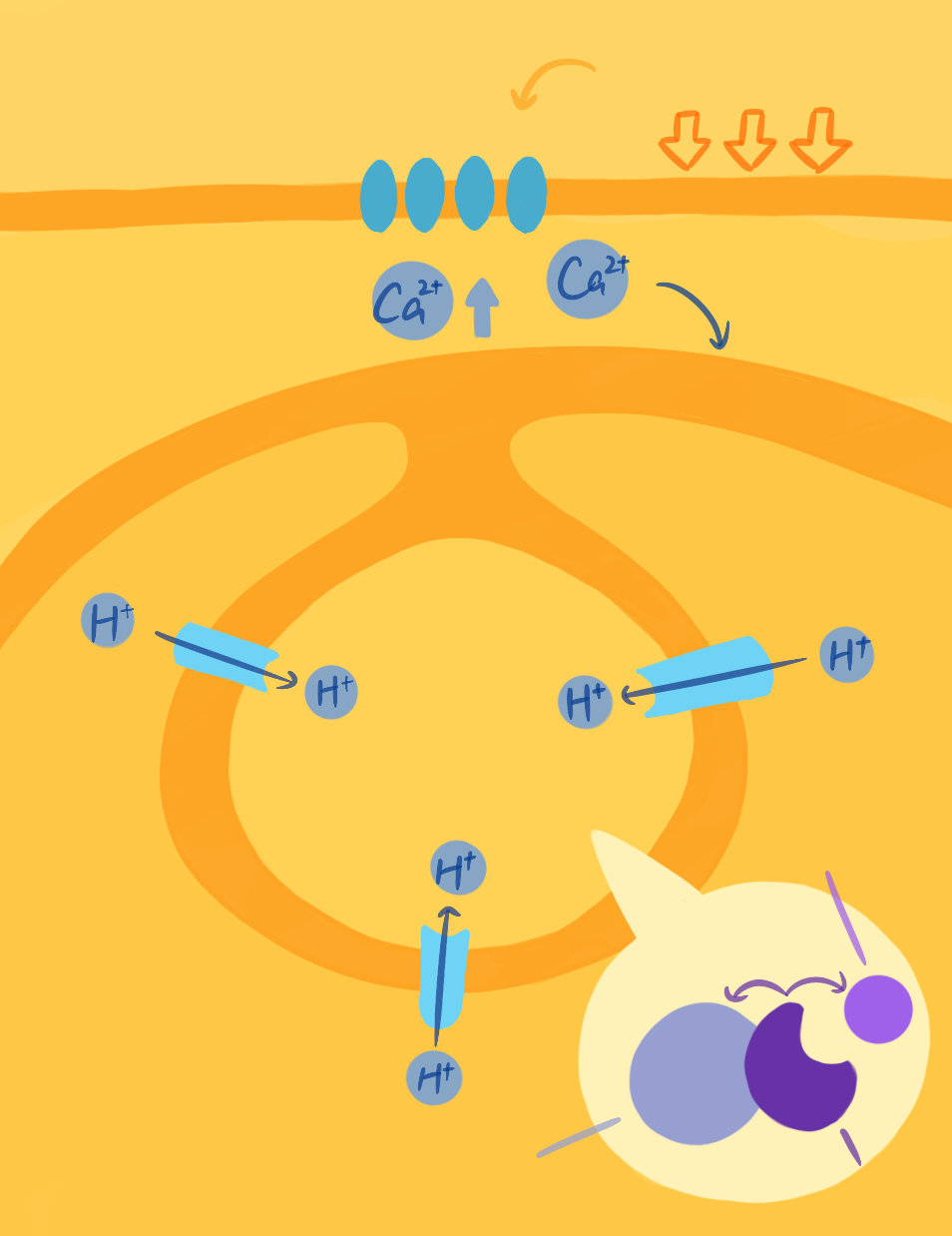
Signal transduction pathway of emitting light.
So how do dinoflagellates emit light? Dr. Michael Latz, a marine biologist at UC San Diego’s Scripps Institution of Oceanography, is interested in elucidating the mechanism and scientific applications of dinoflagellate bioluminescence. Specifically, he seeks to understand how external forces such as water flow are converted into cellular chemical signals that ultimately result in the chemical reaction between luciferin and luciferase, producing luminescence.
Luciferin is a generic term for a class of light-emitting compounds that exist in bioluminescent organisms. These compounds typically undergo an enzyme-catalyzed reaction with oxygen that produces an excited state luciferin intermediate that emits energy in the form of visible light when decaying back to the ground state. This light emitted during the decay process creates the organism’s bioluminescent emission. The enzyme that catalyzes the luciferin oxidation reaction is a protein called luciferase. In dinoflagellates, both luciferin and luciferase are stored in vesicles called scintillons. In order to only emit light upon stimulation, luciferin is typically bound to the luciferin binding protein, which protects luciferin from oxidizing and thus keeps it inactivated inside the scintillons until the activating stimulus is received.
The production and activation of luciferin is also tightly regulated within dinoflagellates. After luciferin is synthesized, it is loaded into scintillons located next to the vacuole, a large storage vesicle inside the cell that contains many protons. The inside of the vacuole has a pH of less than 6, while the pH of the scintillons is more than 8.0, which creates the proton gradient that is crucial for the activation of bioluminescence. Interestingly, the membrane of the vacuole is electrically excitable, similar to a nerve cell. When the vacuole membrane is depolarized, a proton-selective channel opens and conducts protons into scintillons, changing the conformation of the luciferase to expose its luciferin binding site and allowing the chemical reaction to proceed.⁴ Additionally, another ingenious structural strategy in dinoflagellates is that scintillons contain all the components necessary for emitting light and stabilizing it: luciferin, luciferase, and luciferin binding protein. The physical proximity among these three compounds make emitting light a rapid, readily accessible process.
The mechanotransduction reaction cascade starts when dinoflagellates sense mechanical forces, such as the force produced by a predator grabbing them or shear stress caused by water flow, which activate GTP-binding proteins embedded in the plasma membrane.¹ Then, transient receptor potential (TRP) channels on the cell membrane will open, allowing both a release of calcium ions from intracellular stores and an influx of calcium ions into the cell from the extracellular space. This increase in intracellular calcium depolarizes the membrane of the acidic vacuole associated with the scintillons. In turn, this depolarization of the vacuole membrane initiates a self-propagating action potential that travels along the vacuole membrane and opens voltage-gated proton channels in the vacuole membrane. Once these channels open, protons flow into the scintillons resulting in a rapid decrease of pH inside the vesicles that stimulates the release of luciferin from the luciferin binding protein and the activation of luciferase.¹ Finally, now that luciferin can bind luciferase, luciferase facilitates the luciferin oxidation, which emits light. Through this complex mechanism, dinoflagellates are able to respond to external mechanical stimuli within 20 milliseconds—an immensely fast response time for a mechanosensing process in any organism.
One of the key players in the transduction pathway, TRP channels, are essential in many sensory system responses in various organisms. Playing a key role in human temperature and pain sensing, TRP channels are also at the center of biological research in recent years. In fact, the Nobel Prize in Physiology or Medicine 2021 was awarded to scientists who first discovered TRP channels. By understanding their function in dinoflagellates, which might be the most ancient organism to have these channels, researchers may get a glimpse into the origins of TRP channel functions in eukaryotes.
Color of Science
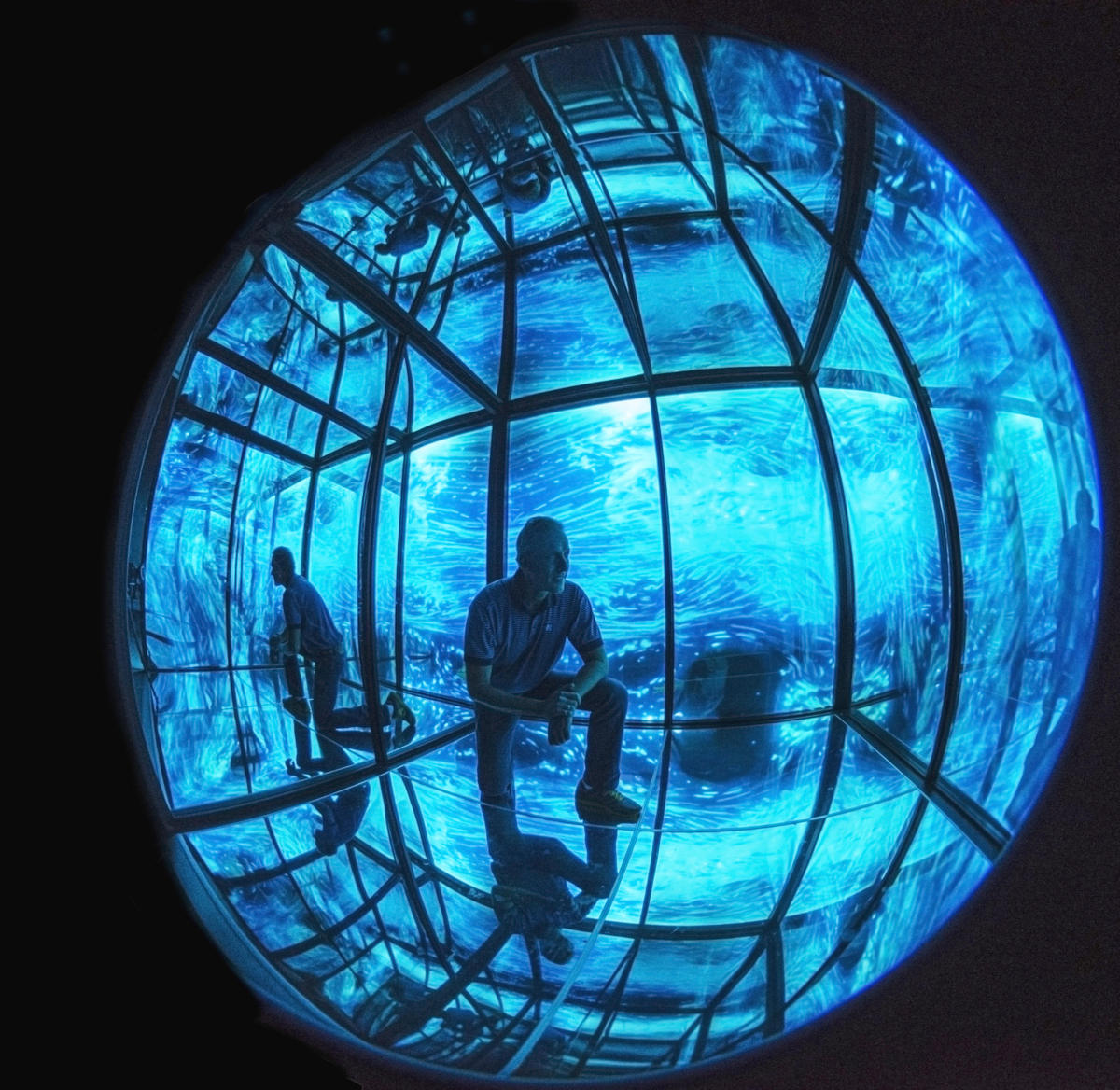
Photo of Dr. Latz in the Infinity Cube exhibit at the Birch Aquarium.
Displays of bioluminescence are known all over the world, from glowing caves in New Zealand to sparkling beaches in the Caribbean and Pacific Ocean. In addition to being visually appealing, light from marine animals has many more real-world applications, such as the use of biofluorescent organism-derived markers like asmCherry (red fluorescent proteins) and GFP (green fluorescent proteins) to stain cells. Unlike bioluminescence, where light is produced from a chemical reaction in an organism, biofluorescent organisms have proteins that absorb light and reemit it at a lower energy level. Dr. Roger Tsien, the late professor of Pharmacology, Chemistry and Biochemistry at UC San Diego and a 2008 Nobel Prize laureate in Chemistry, along with Dr. Shimomura and Dr. Chalfie, drastically increased GFP’s fluorescent signal intensity and photostability.⁵ Since then, GFP has become a mature and popular tool for microscopy.
Similar to the fact that GFP can be used for visualization, dinoflagellate bioluminescent proteins can be used to image flow fields including breaking waves, flow over seabeds, and boundary flows of moving animals and ships in the ocean.¹ Since dinoflagellates can sense flow velocity change and respond to it almost instantaneously by emitting luminescence, they are very useful in visualizing moving animals. Additionally, researchers have used dinoflagellates to track dolphin movement and predict the motion of moving ships for various purposes.
Another application of bioluminescence is to raise peoples’ awareness of the connection between humanity and nature. In 2018, Dr. Latz collaborated with artist Iyvone Khoo to put together a bioluminescence exhibition called Infinity Cube at the Birch Aquarium at Scripps. Khoo was inspired to create the exhibit by a midnight walk at a beach in Mexico where she witnessed the co-shining of the Milky Way and the blue tides that pushed her to ponder about the microcosm and macrocosm, with humans right in the middle. Partnering with Dr. Latz, she composed her unique exhibit: a reflective cube with a media projection of dinoflagellate bioluminescence. Visitors were able to walk into the cube and immerse themselves into a marvelous ocean of blue lights to experience nature as their only illumination in the boundless darkness.⁶ Bioluminescence demonstrations not only engage the public but also provide an opportunity to learn about the wonders of bioluminescence and its role in nature.
Glance into Future
The ability of dinoflagellates to bioluminesce is a true wonder; however, not all dinoflagellates possess this unique capacity. According to Dr. Latz, only about 18 out of 130 total dinoflagellate genera emit light, which raises the question of what makes the bioluminescent genera so special? To answer this question, the Latz group plans to search for the unique genes that code for proteins which enable bioluminescent species to shine. However, one challenge to answering this question is related to a peculiar property of dinoflagellates: their genome is hundreds of times larger than the human genome. Nevertheless, Dr. Latz is confident that with the incredibly fast advancements in molecular biology and genetics, soon it will not be a daydream to have the proper molecular tools to manipulate dinoflagellate genetic expressions, which would help us to confirm the role of related proteins in the bioluminescent signaling pathway inside those shining blue stars of the ocean.
References
- Jenny L, Michael IL. Bioluminescence in Eukaryotic Microbes. Encyclopedia of Microbiology. 4, 526-535 (2019).
- E. J. Buskey. Swimming pattern as an indicator of the roles of copepod sensory systems in the recognition of food. Marine Biology. 79, 165-175 (1984).
- Ali HA. et al. The Influence of the Toxin Producing Dinoflagellate, Alexandrium catenella (1119/27), on the Feeding and Survival of the Marine Copepod, Acartia tonsa. Harmful Algae. 98, (2020).
- Juan DR. et al. Identification of a vacuolar proton channel that triggers the bioluminescent flash in dinoflagellates. PLoS One. 12(2): e0171594 (2017).
- Roger H, Andrew BC, Roger YT. Improved green fluorescence. Nature. 373, 663–664 (1995).
- Michael IL. The Making of Infinity Cube, a Bioluminescence Art Exhibit. Bulletin. 28(4), 130-134 (2019).
Written by Angela Wang
Angela is a Neurobiology Major from Seventh College. She will be graduating in 2023.