Understanding the processes of refined limb movement and developing devices that harness our neural circuits
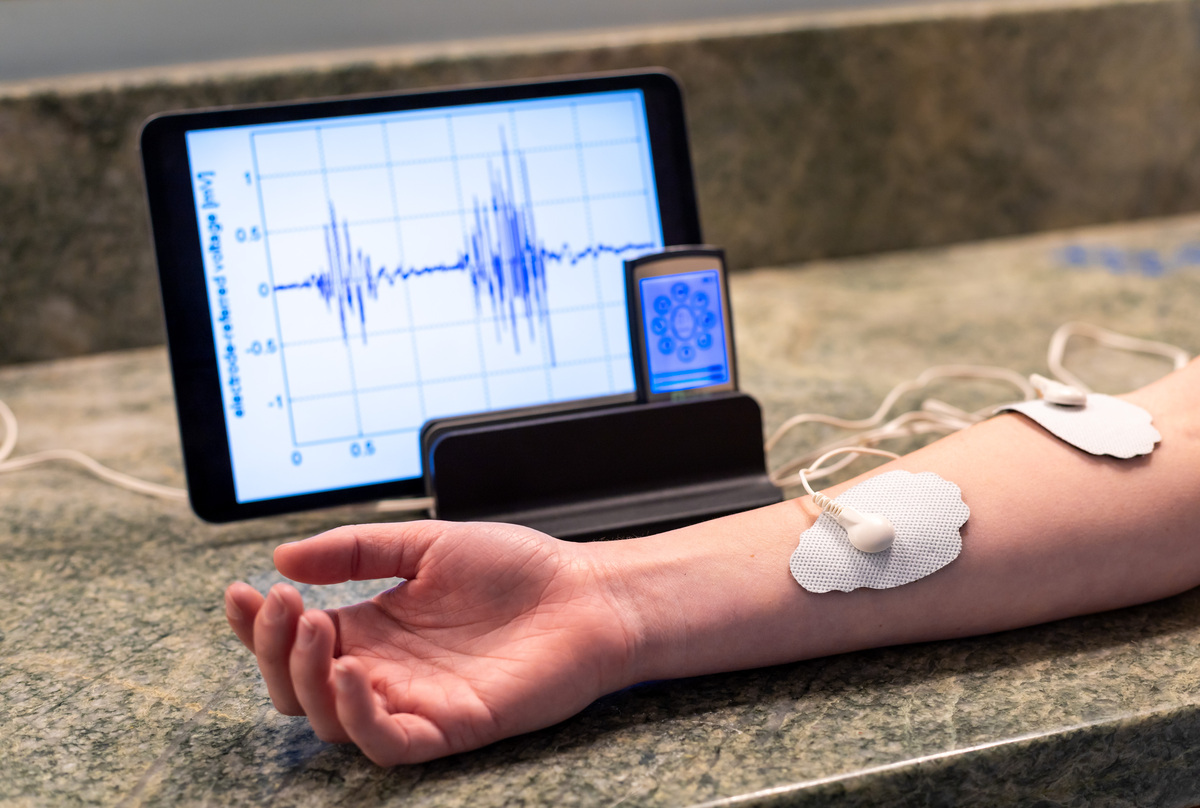
Electromyography (EMG) sensors measure electric signals given off by muscle movement, which the Xu lab can translate into robotic movement.
PHOTO BY SAM ZILBERMAN
Imagine yourself reaching for a pencil, walking to your morning class, or even flipping a page of this publication. Do these actions require your focus, or do you hardly think about them at all? While these simple motions may seem like second nature, they are actually quite complex when considering the millions of neurons and hundreds of muscles necessary for movement. Scientists have barely scratched the surface of characterizing the neuroscience behind motion, but two laboratories at UC San Diego are currently delving deeper into this topic. By looking into different aspects of the neurobiology behind motion, these researchers can gain a better understanding of these intricate processes that could allow for great strides in biological devices, prosthetics, and other movement technologies.
THE NEUROLOGY BEHIND MOTION
At the lab of Dr. Eiman Azim, researchers are exploring the neurobiology of movement by analyzing neural circuits in the brain. Nick Baltar is a first-year medical student who worked in the Azim Lab for over three years, first as an undergraduate research assistant and later as a lab technician. The premise of the lab includes observing motor feedback loops, which are the neural pathways between muscles and the brain that control refined limb movement. One type of feedback loop they found was faster than a classical neural circuit going through the cortex of the brain would be. However, it was more complex than spinal cord reflexes, in which neural circuits remain within the vertebrae for quick reactions and do not extend to the brain.
Researchers hypothesized that this motor feedback loop was correlated to a section of the brain known as the lateral reticular nucleus (LRN), located near the base of the brain. Baltar’s work involved putting this hypothesis to the test. To understand the structure of the LRN better, gene sequences from single cells in the LRN were analysed in order to identify gene candidates where expression rates diverged across different regions of the nucleus. From this pool, he used a fluorescence in-situ hybridization protocol to establish the location of the possible genes that could be influencing these feedback loops in the chromosome. Additionally, Baltar utilized a method of manipulating genes in mice cells called cre-lox recombination in order to better understand the function of different parts of the LRN. After repeating these procedures for ten different candidate genes, he found genes HTR2C and PCP4 had specific expression beneath and to the side of the LRN, the portion of the brain the Azim Lab originally thought to be involved in motor feedback loops. He compared the movement of mice with manipulated DNA to the movement of mice with unaltered DNA in order to observe the effect of the altered DNA on the mice’s movement. The Azim Lab continues to develop on Baltar’s finding, investigating these gene’s involvement with motor feedback loops and complex movement.
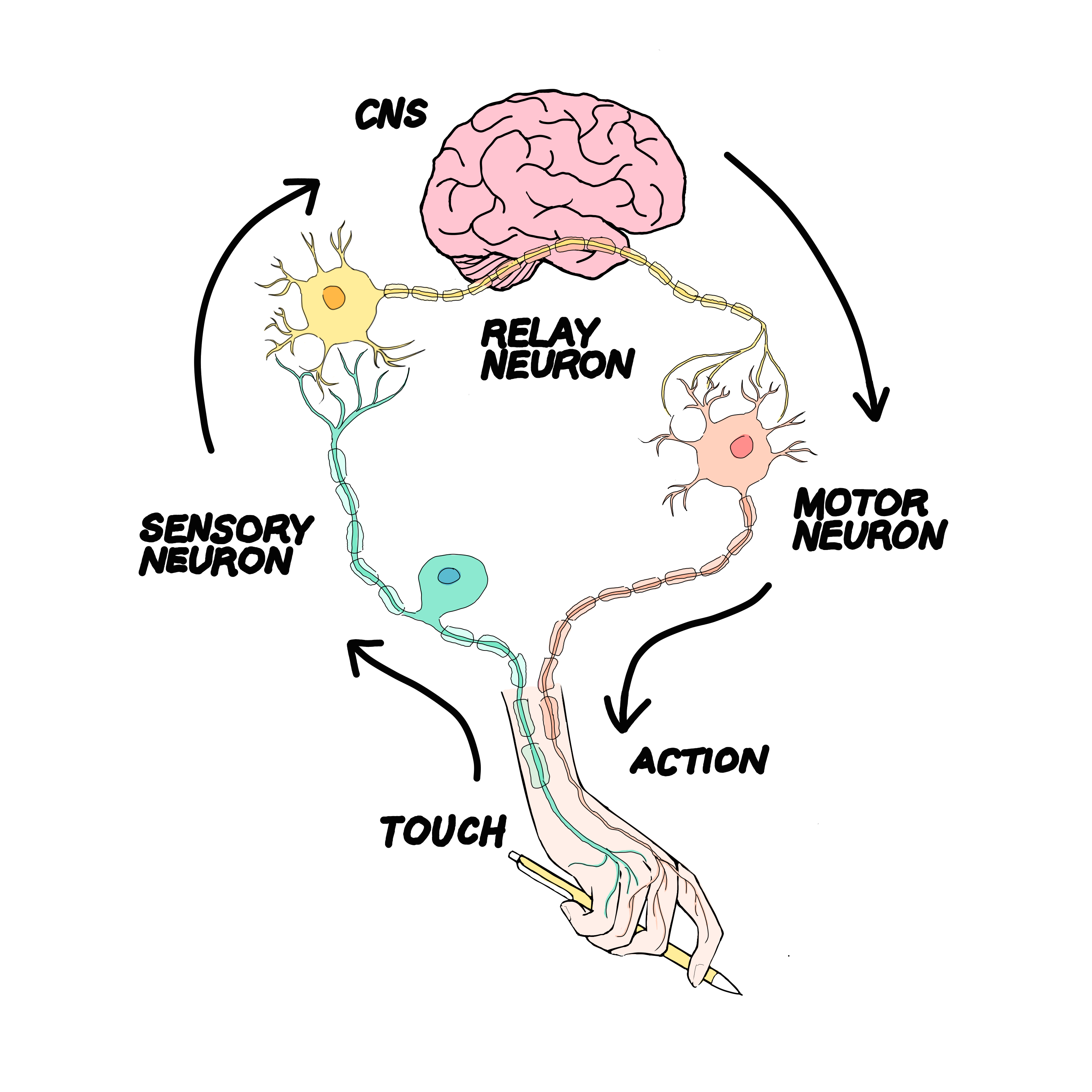
The body and brain communicate through the central nervous system (CNS) to dictate our movement. More specifically, the lateral reticular nucleus (LRN) has been found to be involved in some motor feedback loops, governing refined limb movement.
BRIDGING THE GAP
Baltar’s research now aims to characterize the structure and function of the complicated neural circuits that allow us to perform movements we hardly think about on a daily basis. However, just like any complicated machine, our bodies can malfunction. What happens when things go wrong? Amputations and spinal cord injuries are examples of incidents that cause people to lose their normal motor functions. Neurobiological research is now paving the way for engineering of devices that can address such situations.
In the Nanoengineering Department at UC San Diego, one group is aiming to bridge the gap between theory and application. In 2018, Dr. Sheng Xu’s lab made the cover of Nature Electronics for developing a small, multilayer, flexible electrical circuit that stretches and distorts without any damage. This design allows the circuit to stick to fabric or skin. Thanks to their novel framework, these non-invasive circuits are now being developed for many different applications in healthcare such as a blood pressure monitoring system.
TURNING NATURE INTO TECHNOLOGY
One of the developing applications of this technology in the field of neurobiology is the use of the electromyography (EMG) sensor, which takes advantage of the electrical signals involved in movement. A tiny chip three centimeters wide and two millimeters thick can be stuck onto skin and transform human movement into robot movement. EMG sensors utilize the electrical signals our brain sends to our muscles via motor neurons; these motor neurons then stimulate muscle fibers, causing them to twitch and elicit contraction of the whole muscle. During contraction, muscles emit electrical signals known as EMG waves. As muscles can conduct electricity in a manner similar to neurons, sensors placed on the skin near muscle are able to measure the amplitude and frequency of these waves. Then, these signals can be gathered and interpreted, before being translated into code and relayed to machines such as a robotic arm, opening the door to many possibilities, such as prosthetics for amputees and those with spinal cord injury, and remote-control robots capable of performing work.
At the Xu lab, two UC San Diego seniors, Amer Yaghi and Geoffrey Hand, are working to bring a small, wearable EMG sensor to life. The circuit they built contains a gyroscope (which can measure orientation), an accelerometer (which can measure acceleration), an EMG sensor, and a Bluetooth transmitter, which all work together to control a robotic arm. Thanks to their lab’s novel 3D approach to stretchable electronics, the team is able to fit a large amount of information into one small patch. In the past, engineers were limited to single-layer circuits, but by fabricating multiple layers of this flexible circuitry and joining them vertically through soldering, it is now possible to layer circuits on top of each other and accommodate many more components. The self-contained system can then wirelessly transmit to a robotic arm through Bluetooth, controlling movements with almost no delay. This technology has implications for prosthetics for amputees and controlling remote work in the future.
When asked how the EMG sensor can translate electric waves into a specific movement to control a robotic arm, Hand says there is not a way for humans to recognize the patterns based on EMG readings by eye. The lab is currently using artificial intelligence guided by deep learning algorithms to determine the meaning of EMG signals. Their program is trained to recognize specific EMG wave patterns associated with certain movements such as grabbing or releasing.
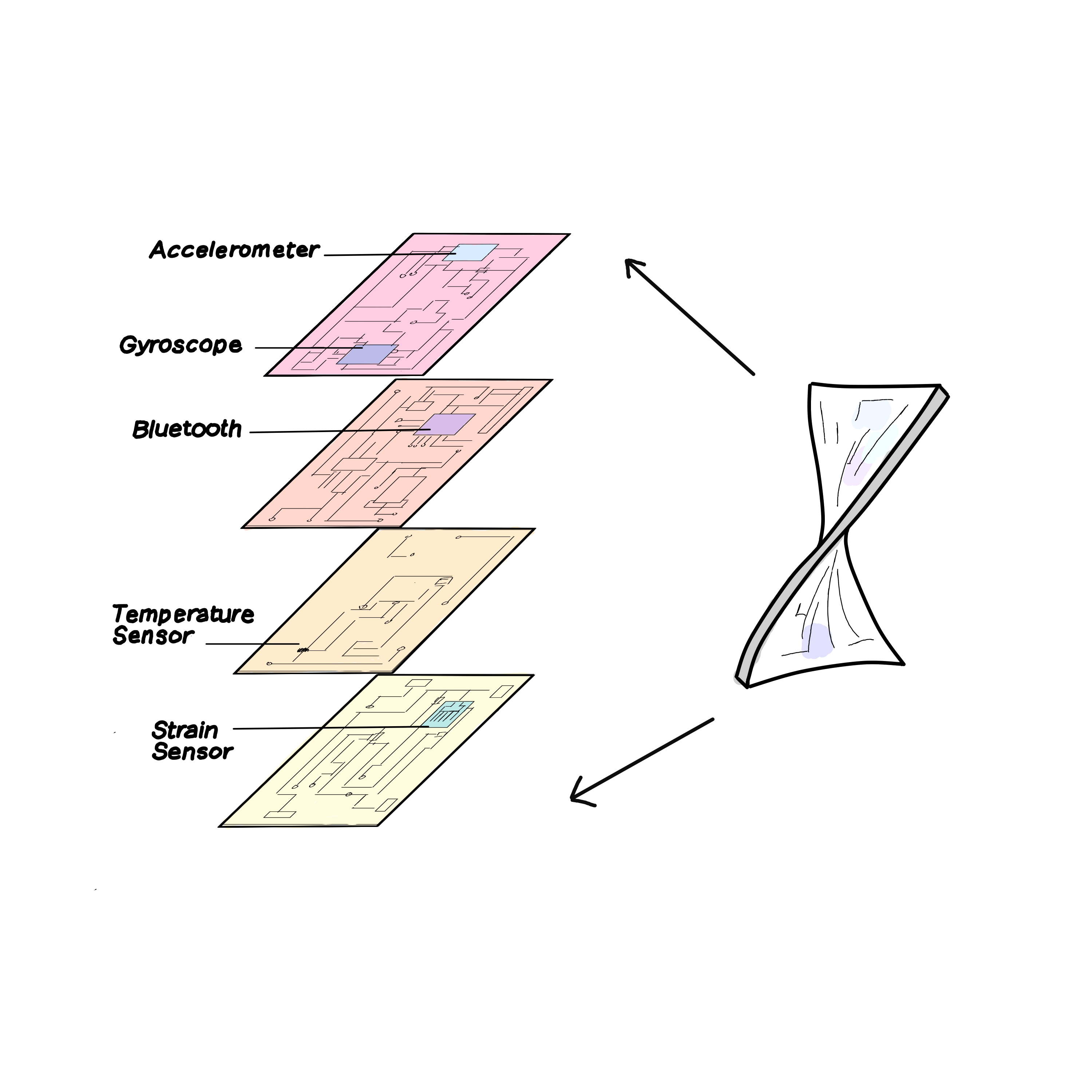
New breakthroughs in nanoengineering allow multiple circuits to be layered on top of each other, increasing the amount of functionalities the small, flexible device can hold.
Another potential application of this technology is to detect fatigue in muscles. Fatigue is sometimes identified by calculating the mean and median frequencies of the EMG waves, with a decrease in these frequencies indicating muscle fatigue. However, this theory is not well established, and Hand is exploring novel metrics and statistical methods which may allow him to better quantify fatigue through more complex analyses of wave frequency.
Although these flexible biosensors are innovative pieces of technology, this project has not come without its difficulties. Yaghi explains that the electronics are delicate and fragile, requiring the handler to be very careful; in fact, they are so tiny that he had to learn how to weld under a microscope. In developing software used to program these types of sensors, the devil is in the details, as Yaghi once spent four weeks debugging a single circuit. Hand agrees that there is always lots of debugging to do, as it is difficult to smoothly connect code to the device. Using flexible silicone rubber instead of a rigid circuit board also opens the door to more obstacles than would be encountered in traditional electronics. Much like Hand and Yaghi’s coding and circuits, motion is a very complicated subject. This developing technology will become useful in the future, as those with limb deficiencies can wear similar devices to control a prosthetic limb that mimics their movements.
LOOKING FORWARD
Unlike the Azim lab, which characterizes specific neural pathways and the structure of the nervous system, researchers in the Xu lab do not have to understand the specific route electricity takes to cause particular maneuvers. Instead, artificial intelligence can be trained to fill this gap and to translate electricity into movement. This is a stark contrast to Baltar’s work in the Azim lab, where he is working to piece together the minute details of how electrical signals are processed and transmitted to perform motions. Baltar admits that the interface between neurobiology and engineering still has a long way to go before being viable for use in robust neural prosthetics, especially when considering the intricate neural circuits he is studying. Since there is some disconnect between the frontiers of engineering and biology, a greater collaboration between the two sides is required to reach a common ground, and both theoretical understanding and its applications are vital in reaching these advanced goals. Those involved in research of motion and neural circuits will always be searching for further understanding. However, new technologies and protocols, such as circuit components and genetic engineering, are constantly allowing for breakthroughs in the field. Through collaborative research between biology and engineering, we can come to better understand how electricity directs motion and work towards solutions for neurobiology’s most challenging questions.
WRITTEN BY ANNIKA SO & EMILY WHITE
Annika So is a second year student majoring in Biochemistry/Chemistry. Emily White is a first year student majoring in Molecular and Cell Biology.
FROM UNDER THE SCOPE VOL. 11
To read the original version, please click here. To read the full version on our website, please click here. To read more individual articles, please click here.